Research
Sonogenetics
We are developing a new approach to control both neurons and non-neuronal cells non-invasively. We have identified specific proteins that render target cells sensitive to low intensity ultrasound stimuli. We have demonstrated the efficacy of our method in both C. elegans and M. musculus models. Please visit our sonogenetics.salk.edu for more information, or to request reagents or initiate collaborations.
Strategic Decision-Making Underlying Foraging Behavior
We investigate how animals make strategic decisions in complex foraging contexts. How do animals integrate relevant external and internal parameters to plan foraging strategies that balance risk and reward? Which factors determine how animals value overall long-term success over short-term food rewards? Foraging strategies are critical for dealing with conditions in which feeding conflicts with another goal, such as exploration, predator avoidance, or territorial defense. However, little is known about how these foraging strategies are implemented. To answer these questions, we design naturalistic behavior paradigms that elicit rich and context-specific responses, build computational models of behavioral algorithms, and finely tune experimental parameters to conduct granular analyses. By studying nematodes with simple nervous systems and powerful genetic tools, we can link molecular and neural mechanisms to the decision processes that guide strategic foraging behaviors. Our work has revealed surprisingly sophisticated and advantageous strategic decision-making in nematodes with only ~300 neurons.
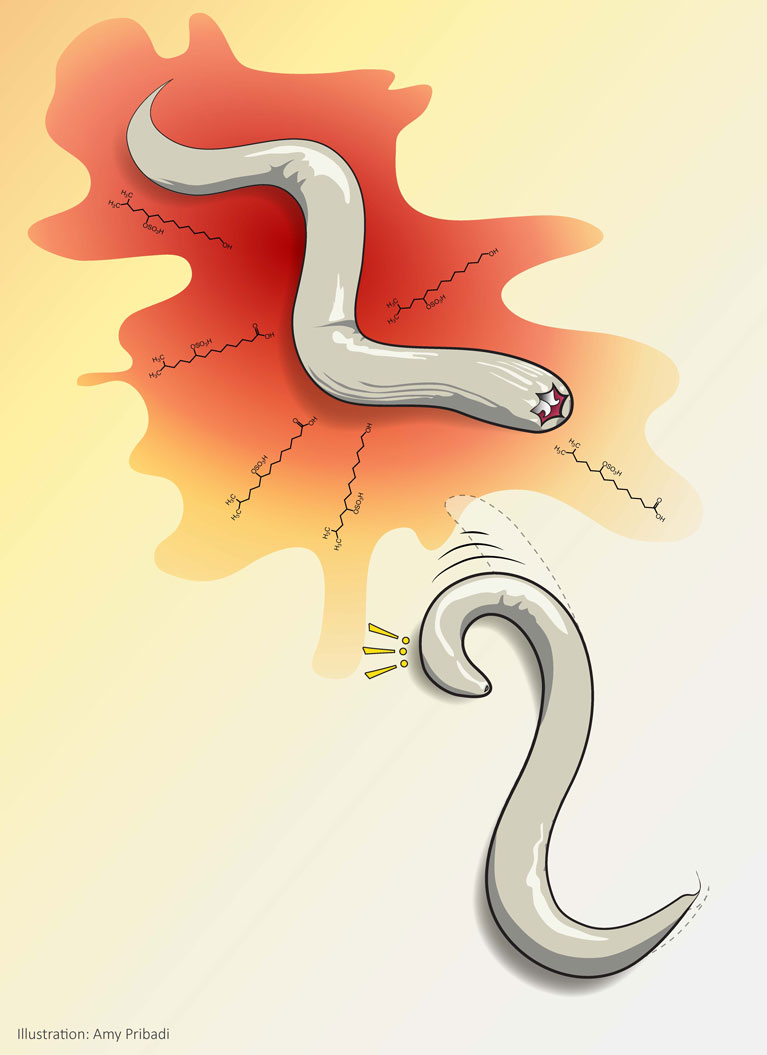
Credit: Amy Pribadi
Predator-prey interactions: The predatory nematode Pristionchus pacificus competes with the prey nematode Caenorhabditis elegans for bacterial food. We use this predator-prey system to study the predator’s response to the prey , as well as the prey’s response to the predator. We have demonstrated that P. pacificus can switch between predatory and territorial foraging strategies when both prey and bacterial food are present, a process mediated by octopamine (invertebrate homolog of norepinephrine). By using neuroeconomics and foraging theory to model the strategic value of biting, we found that P. pacificus is motivated by predation when it bites larval C. elegans (easy to kill, low competitive threat), and by territorial defense of bacterial food when it bites adult C. elegans (difficult to kill, high competitive threat). We also study how C. elegans adjusts its foraging strategy when it encounters both food and predators. While we have previously shown that C. elegans exhibits an escape response to sulfolipids in P. pacificus secretions, we are now interested in understanding how the experience of being bitten on a predator-inhabited bacterial food patch leads to changes in C. elegans behavior.
Searching for food: How does prior experience affect how C. elegans searches for food? Our work has shown that C. elegans uses dopamine to encode spatial variability within a food patch, and then uses this experienced variability to influence search behavior after being relocated to a food-less environment. We found that C. elegans searches a wider area of the food-less environment after experiencing high food variability, and searches a smaller area after experiencing low food variability. This is consistent with a maximally informative strategy that considers the area that would have to be explored to accumulate sufficient evidence that the current food-less environment is different from the prior food experience. In addition to how C. elegans searches for food in a food-less environment, we also study how C. elegans chooses between exploitation or exploration when many food patches are available.
Whole-Brain Imaging & Sensory Information Processing
With an optically transparent body, small body size (~1 mm), and only 302 neurons, C. elegans is well-suited for whole-brain imaging. We record from the bulk of neurons in the C. elegans head to gain insights into how sensory information is processed by the brain at the network level. To precisely deliver complex sequences of stimuli, we use microfluidic devices that can rapidly alternate between delivery of different laminar flows of liquids over the C. elegans nose. We employ a variety of computational methods to relate this high-information sensory presentation to whole-brain activity, with the goal of uncovering how sensory input is transformed into network perturbations. We recently developed a fully interpretable model that dissects how neural populations work together to produce state dependent sensory gating at the motor and command neuron level. Using this model, we identified states in which motor and command neurons were either receptive to or largely unperturbed by food stimuli. Furthermore, we were able to deconstruct how stimulus features, such as stimulus timescales and sensory context, differentially affect state-dependent sensory gating. In other work, we used a graph theoretic approach to identify network interaction patterns that are altered when exposed to various attractants and repellents. We used a machine learning classifier to show that graph theoretic features perform better than simple statistics (averages, standard deviation, etc.) in identifying specific sensory stimuli, particularly salt. We are now expanding our research scope to probe how sensory information is processed in freely moving animals.
Credit: Jess Haley